Developing energy-efficient lubricants and coatings for automotive applications
Dr. Boris Zhmud | TLT Feature Article September 2011
Manufacturers are stepping up their R&D efforts to build better engines and lighter materials.
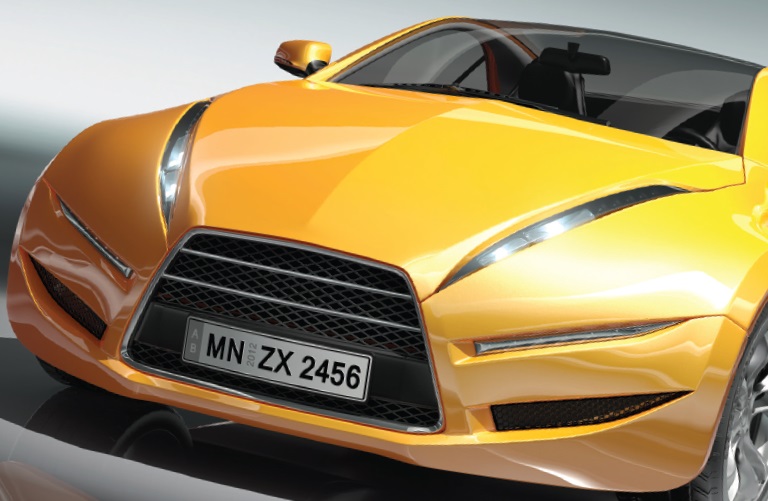
www.canstockphoto.com
KEY CONCEPTS
•
Friction and wear can be controlled by using materials, coatings and lubricants.
•
Improving power transmission efficiency can help achieve better fuel economy.
•
Antifriction coatings are known to reduce friction, minimizing dependence on additive packages.
Editor’s Note: Portions of this article were published with permission from
Lube magazine.
Auto manufacturers are facing increased pressure because of new automobile fuel economy standards enacted by governments in the G-20 major economies and high fuel prices. Thus, the U.S. EPA is looking at standards for 2017 and beyond, setting a target of 62 mpg by 2025. However, according to a recent article published in
The Detroit Free Press, this standard likely will be lowered to 56 mpg.
Because of political and economical incentives, major OEMs are strengthening their R&D efforts in improving fuel efficiency. On the engineering side, alternative energy sources are reducing greenhouse gas emissions, new materials are lowering vehicle weight, and hybrid cars are optimizing power train efficiency. Meanwhile, there is renewed emphasis on understanding the tribological aspects of energy losses in powertrain and utilizing current advancements in lubrication engineering and coatings to minimize those losses.
Following are three ways to control friction and wear:
•
Materials. Choose lighter and durable materials with appropriate mechanical and tribological properties to manufacture mechanical parts.
•
Coatings. Improve tribological behavior of existing materials by means of surface coatings.
•
Lubricants. Develop lubricants to obtain desired tribological behavior for a given material.
Development, material and production costs are always important factors to consider when the market potential for any of these approaches is assessed.
SMARTER ENGINES AND LIGHTER CARS
In the past decades, engineering advancements in manufacturing have not come unnoticed. The average fuel consumption, normalized to engine output, dropped from 10 L/100 km (23.5 mpg) in the 1980s to 5L/100 km (47 mpg) because of the broad acceptance of fuel-stratified injection (FSI) direct-injection technology. Though FSI technology has been around for at least half a century, its advantages could not be fully realized until electronic engine control modules became available. FSI technology increases the torque and power of spark-ignition engines, making them as much as 15% more economical at a given power output.
The auto industry in Europe and North America has now switched completely to direct fuelling as it has introduced new petrol engines. The majority of modern FSI engines are actually turbo-FSI (TFSI or TSI) as they combine direct injection with twin-charging, which is a turbocharger and supercharger working together.
In a FSI engine, the fuel is injected into the cylinder just before ignition. This allows for higher compression ratios without knocking and leaner air/fuel mixtures than in conventional Otto-cycle internal combustion engines. By regulating injection pressure/valve timing and lift, constant electronically aided engine efficiency tuning is possible based on the actual load, fuel type, exhaust parameters and ambient conditions.
An alternative to FSI is homogeneous-charge compression ignition (HCCI) technology, which can be viewed as a hybrid of homogeneous-charge spark ignition (in gasoline engines) and stratified-charge compression ignition (in diesel engines). In theory, HCCI allows one to achieve gasoline engine-like emissions along with diesel engine-like fuel efficiency. Analogous to diesel engines (in an HCCI engine), the air/fuel mixture is ignited due to compression without using an electric discharge. Stratified-charge compression ignition in diesel engines also relies on temperature and density increase resulting from compression, but combustion occurs at the boundary of fuel-air mixing caused by an injection event to initiate combustion.
Inherently, for HCCI engines, transient control is more difficult than for other modern IC engines, and to date there have only been few prototype engines running in the HCCI mode. A combination of spark-assisted and HCCI combustion can be a perspective development path. Recently, a vehicle powered by a 25-cc, 1.3-hp HCCI engine deploying WS
2 antifriction coating was constructed by Royal Institute of Technology KTH in Stockholm, Sweden, as shown in Figure 1.
Figure 1. Agelis ecocar built at Royal Institute of Technology KTH in Stockholm, Sweden managed to run for 481 km at 1 L of gasoline during Shell Eco Marathon 2010. Critical engine components had antifriction coatings made by Applied Nano Surfaces, and a fuel economy engine oil produced by Elektrion s.a. was used.
Significant progress has occurred in the development of diesel engines following the introduction of high-pressure common-rail direct injection, variable geometry turbochargers and charge-air intercoolers during the past two decades. While thermal management is of increasing research interest, some novel diesel engines (e.g., Volkswagen’s three-cylinder TDI installed on Lupo and top-notch V10 TDI) have deployed a particularly rigid aluminum crankcase with an innovative gray cast-iron bearing tunnel in order to achieve low noise levels in a low-weight design. The adoption of an aluminum cylinder block in the diesel engine promoted development of plasma-spray coating technology, whereby a thin layer of cast iron is deposited onto the surface of the cylinder walls, greatly improving their mechanical strength and wear resistance.
Improving power transmission efficiency can help achieve better fuel economy, and consequently, continuously variable transmissions and automatic gearboxes with six to eight speeds are becoming increasingly common.
In order to keep costs down, manufacturers are developing new materials such as advanced ultra high-strength steel (A-UHSS) to reduce vehicle weight and increase passenger safety.
(Note: You can learn more about A-UHSS in the July 2011 issue of TLT, available digitally at www.stle.org). While aluminum engine blocks have become the standard in passenger cars and other small vehicles, the move to aluminum was primarily motivated by curb-weight reduction. For example, the body parts of some luxury cars (e.g., the Audi A8 and R8, Jaguar XJ and BMW 7) are made of aluminum, which offers improved vehicle performance. In addition, the body parts of high-priced sport cars such as Koenigsegg, Bugatti and Lamborghini are made of carbon fiber or carbon-fiber reinforced plastics (CFRP).
Another noteworthy advancement on the material side is the use of lightweight porous metals and composites as impact energy absorbers and sound-damping elements.
ANTIFRICTION COATINGS
In an internal combustion engine, around 15% of energy is lost due to friction, (
1, 2) as shown in Figure 2. This 15% can be further subdivided, in a proportion 9:1, into a dissipative part (viscous dissipation due to lubricant flow) and a frictional part (mostly due to boundary friction in piston ring/cylinder bore, cranktrain and valvetrain systems). The dissipative losses can be reduced by using lower-viscosity oils and smaller displacement volumes. The frictional part can be reduced by using antifriction coatings on performance-critical parts, as well as by deploying special friction-reducing additives in engine oil.
Figure 2. Approximate distribution of energy losses within the internal combustion engine.
Unfortunately, the use of additives in oil may cause exhaust catalyst poisoning and must be constrained. Thus, the phosphorus content in ILSAC GF-5 engine oils must not exceed 800 ppm. This makes coatings an attractive alternative, minimizing the dependence on additives.
Nowadays, various coatings are used in automotive engineering to compensate deficiencies of bulk materials. Coatings can be used to improve wear resistance, corrosion resistance, appearance, adhesive properties, etc. For instance, Nikasil, Alusil or wire-arc sprayed iron coatings are used for reinforcement of cylinder bore walls and improved oil film retention in aluminum engines. Other classical methods used for enhancing the tribological properties of various automotive components are chrome plating, ferritic nitrocarburation and phosphatation.
From an automotive engineering perspective, whenever advancements in coatings are discussed, one often tends to focus exclusively on hard antiwear coatings such as diamond-like carbon (DLC), boron nitride (BN), silicon carbide (SiC), titanium nitride (TiN), tungsten carbide (WC), etc. This is probably explained by the fact that, from the car owner’s perspective, an engine that wears prematurely is a much worse choice than a robust engine that has marginally higher fuel consumption.
The development of hard coatings started in the 1960s with chemical (CVD) and physical (PVD) vapor-deposition techniques. There are many PVD variants in use today (magnetron sputtering, evaporation by laser, wire arc, electron beam, etc.) Hard coatings have many unique properties such as chemical inertness and extreme resistance against abrasion, making them invaluable in the tooling industry. Notwithstanding their impressive antiwear performance, hard coatings help little to improve fuel economy. The fact that DLC coatings afford a reduction of the coefficient of friction from 0.3 to 0.15 in a dry steel vs. steel contact does not mean one is going to enjoy a 50% friction reduction in an engine where all moving parts are lubricated and the characteristic value of the coefficient of friction is already well below 0.1. As a matter of fact, hard coatings may increase friction by inhibiting the effect of lubricity additives in oil.
The goal of antifriction coatings is to reduce friction, thereby minimizing dependence on the additive package. In an attempt to combine the mechanical toughness of hard coatings with high lubricity, composite PVD coatings, which exhibit self-lubricating properties, such as Balinit C (WC/C, Balzers Ltd.) and MoST (MoS
2/Ti, Teer Coatings Ltd.) have been developed.
Soft-sacrificial coatings represent a fundamentally different philosophy in the development of antifriction and antiwear coatings, as the coating can be sacrificed in action while protecting the coated parts.
For example, molybdenum disulfide (MoS
2) coatings were pioneered by Alfa Molykote after World War II. After acquiring Molykote in 1964, Dow Corning developed and manufactured a few lines of Molykote solid lubricant coatings. Molykote coatings are based on MoS
2 as the main friction-reducing component, but they may contain a number of other ingredient such as graphite, resin binder, corrosion inhibitor, etc., which are required to control consistency, adhesion, corrosion resistance, appearance and other properties. A similar concept has been used in the development of EcoTough coatings for piston skirt by Federal-Mogul Corp.
The EcoTough coating consists of a blend of graphite, carbon fibre and molybdenum disulfide bound in a resin binder, and is claimed to provide superior friction reduction and scuff resistance. Tungsten disulfide (WS
2) is another member of layered transition metal dichalcogenides (LTMD) used as a substitute for MoS
2 in the aeronautics and spacecraft industry. As compared to MoS
2, WS
2 has superior high-temperature performance and is less sensitive to humidity. Applied Nano Surfaces (ANS) has pioneered a revolutionary new technology for friction and wear reduction using WS
2-based tribocoatings.
The ANS technology (known as the ANS triboconditioning method) is a dedicated metalworking/finishing process that combines elements of extreme-pressure mechanical burnishing of the component surface with a tribochemical or mechanochemical deposition of a low-friction antiwear film of WS
2. The mechanical treatment is essential for improving the surface finish by leveling off asperities and building up compressive stresses within the underlying material and for initiating the triboreaction, leading to the in situ formation and interfacial nucleation of tungsten disulfide onto the surface.
When applied to components made of steel or cast iron such as cylinder liners, camshafts, piston pins, gears, etc. (
see graphic), the ANS process significantly improves their tribological properties. The primary effects are a reduction in wear and a shift in the Stribeck diagram down (WS
2 effectively reduces boundary friction) and to the left (removal of asperities extends the full film lubrication towards higher loads), as shown in Figure 3.
Figure 3. Changes in the surface topography and tribological properties of the cylinder liner surface induced by the ANS triboconditioning process: (a) cylinder liner and liner segments used in the tests, (b) SEM images of the honing structure and (c) friction between liner and piston ring measured using a reciprocating test rig (shown on top) and the bearing curves of surface roughness (shown on bottom).
As a result, the coefficient of boundary friction is reduced by 20% to 60% while wear is reduced by 4 to 10 times. Other benefits include improved surface finish and surface integrity, reduced tribomutation and fatigue accumulation during the running-in period, compressive stress build-up and improved lubricant film strength.
The tribocoatings generated by the ANS triboconditioning process had exceptionally strong adhesion to the component surface. No coating delamination was observed in the scratch test until a cohesive failure or plastic deformation in the substrate material occurred. The outstanding wear resistance of ANS-coated parts allows one to switch to lower viscosity lubricants for improved energy efficiency without accruing risk of wear-related failures.
ENERGY-EFFICIENT LUBRICANTS
Since a significant part of energy losses in the internal combustion engine comes from viscous dissipation, the trend has shifted toward low-viscosity oils from SAE 40 and 50 in the 1960s-1980s to current SAE 20 and 30 viscosity grades. This transition has been facilitated by availability of high-quality hydroprocessed and synthetic base oils (
3).
Due to their greatly reduced volatility and good low-temperature performance, modern base oils of API Group II-IV allow the formulation of thinner engine oils of 0W-30, 0W-20 or even lighter grades to achieve better fuel economy. As shown in Table 1, use of lower viscosity engine oils significantly reduces energy losses in the main bearing and piston/bore systems, while losses in valvetrain increase due to boundary friction. This phenomenon is depicted in Figure 4. By decreasing oil viscosity, one decreases energy losses due to viscous dissipation while at the same time increasing losses due to boundary friction.
Table 1. Estimated energy losses in different engine subsystems depending on engine oil viscosity. Mercedes Benz M111 2.0L gasoline engine at 2,500 rpm (Taylor, Proc. IME 211 (1997) 235).
Figure 4. Dependence on high-temperature, high-shear viscosity of boundary friction and viscous dissipation components contributing to the mechanical energy loss in the internal combustion engine (shown on top) and of engine wear rate (shown on bottom). A balance between frictional and viscous losses is believed to be achieved in the HTHS viscosity range of 2.2 to 2.8 mPa s. However, in order to eliminate the risk of wear-related failures and to cover a variety of engine designs, engine oils with HTHS viscosity above 3 mPa s are preferred in practice.
This makes a strong argument for deploying friction modifiers, of which molybdenum phosphothioates are the most common in formulations of fuel-economy engine oils. However, development of a balanced formulation is not as straightforward as it appears, and numerous pitfalls may be encountered. For instance, though efficient for valvetrain protection, molybdenum derivatives may cause bearing corrosion problems. Another big hurdle is that certain additives require high-treat levels for fully revealing their tribological effect, and such high levels are not acceptable due to potential negative impact on emission control equipment. Finally, there is always a cost factor.
Extreme pressure antiwear (EP/AW) additives reduce friction and wear by chemically reacting to the metal surface under boundary contact conditions, yielding a reaction product that prevents cold welding. Unlike conventional EP/AW additives such as sulfurized olefins, tricresylphosphate and zinc dialkyldithiophosphate, which chemically react with metal surfaces when a direct asperity-asperity contact occurs in the boundary lubrication regime, boundary-lubricity additives function by physical adsorption onto the rubbing surfaces. In other words, boundary-lubricity additives reduce friction and wear by forming adsorbed surface layers (fatty amides, esters) or slippery surface deposits (graphite, Tefl on, MoS2), which physically separate the rubbing surfaces from each other, while EP/AW additives start to act after the asperity-asperity contact has occurred, but they do not prevent its occurrence.
Boundary-lubricity additives keep their lubricity-enhancing effect even if there is no reciprocal motion between the rubbing surfaces. That is why they are so efficient in controlling stick-slip and chatter phenomena. It is important to realize that many additives are multifunctional. For instance, sulfurized olefins, borate esters and phosphate esters have both boundary lubricity and EP/AW functions.
One special class of boundary-lubricity additives falls outside the existing classification—surface-gel-forming friction modifiers or superlubricity additives. Examples include certain amphiphilic ester-based comb-copolymers and Elektrionised vegetable oils (
4). These additives form a sponge-like viscoelastic surface layer retaining the base oil in the tribocontact even at zero sliding speed (zero Hersey number), thus expanding the range of operating conditions under which film lubrication is sustained.
Superlubricity additives build upon the concept of biomimetic lubrication. A good example of a superlubricity effect is walking on slippery rocks on the seashore. What makes those rocks so slippery is the algae slime growing on the rock surface. The algae slime retains a sufficiently thick layer of water between your feet and the rock surface to enable transition from boundary to film lubrication regime under the pressure (equal to your body weight divided by the area of your footstep) when water alone would fail to provide adequate film strength.
As shown in Figure 5, by shifting the Stribeck curve to the left, friction modifiers cause an equivalent shift of the wear and the frictional losses curves in Figure 4. The result is that the optimal viscosity range (shaded in blue) corresponding to the greatest fuel economy is shifted to the left and fuel efficiency is improved.
Figure 5. Stribeck diagram comparing the tribological effect of conventional EP/AW and boundary-lubricity additives with the effect of superlubricity (SL) additives forming gel-like surface layers. (μ) – the coefficient of friction, (η) -viscosity, (v) - sliding velocity and (p) - applied pressure. High pressure and low sliding velocities force the tribosystem into the boundary lubrication regime in which most intense friction and wear occur. EP/AW additives, as well as regular boundary-lubricity additives, shift the Stribeck curve down, reducing friction in the boundary lubrication regime. Superlubricity additives shift the Stribeck curve to the left, maintaining the film lubrication regime over a broader range of tribological conditions.
The ANS triboconditioning process allows one to achieve the same result without having to use friction modifiers in engine oil. Without the ANS process, rubbing parts in an engine would be triboconditioned or run in during the engine operation under conditions which are far from optimal. The major difference that the ANS process brings is that breaking-in of engine components becomes a part of the component manufacturing process. Among other benefits, this greatly reduces dependence on EP/AW additives in oil, eliminating the need to compromise between the level of antiwear protection and the lifetime of exhaust catalyst.
CONCLUSIONS
To summarize, there have been three major developments leading to improvements of fuel efficiency in automobiles in the past decades:
•
Powertrain optimization and curb-weight reduction
•
Use of energy-efficient lubricants
•
Use of antifriction coatings.
REFERENCES
1.
Taylor, R.I. and Coy, R.C. (1999), “Improved Fuel Efficiency by Lubricant Design: A Review,”
Proc. Inst. Mech. Eng.,
214, pp. 1-15.
2.
Green, J.H., Priest, M., Morina, A. and Neville, A. (2003), “Approaches to Sensitising Engine Valve Train Friction Models to Lubricant Formulation Characteristics,” in Tribological Research and Design for Engineering Systems (D. Dowson et al., Eds.), Elsevier, Amsterdam, pp. 35-45.
3.
Zhmud, B. and Roegiers, M. (2009), “Base Oils Pose a Challenge for Solubility and Lubricity,” TLT,
65 (7), pp. 34-39.
4.
Roegiers, M. and Zhmud, B. (2009), “Tribological Performance of Ionized Vegetable Oils as Lubricity and Fatty Oiliness Additives in Lubricants and Fuels,”
Lubrication Science,
21, pp. 169-174.
Boris Zhmud is chief technology officer for Applied Nano Surfaces Sweden AB in Uppsala, Sweden. You can reach him at boris.zhmud@appliednanosurfaces.com.