Biomimetics: The science of imitating nature
R. Arvind Singh, Eui-Sung Yoon & Robert L. Jackson | TLT Cover Story February 2009
Tribologists are learning from some of the natural world’s most advanced engineers, including the lotus plant, gecko, tree frog, shark, pangolin and a host of others.
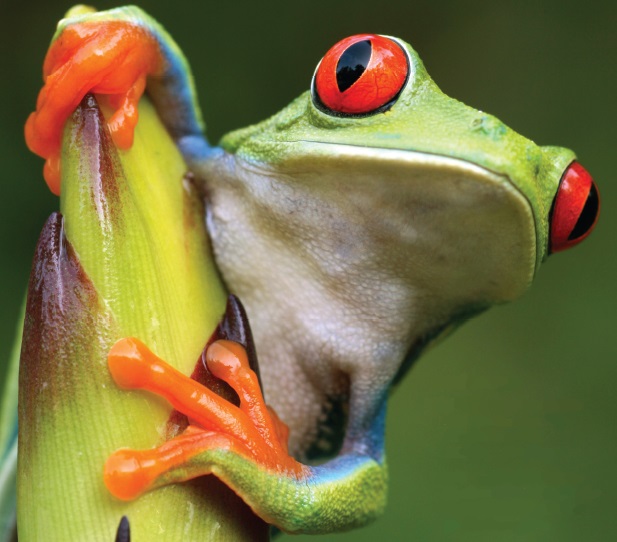
www.canstockphoto.com
KEY CONCEPTS
•
Already popular in materials science and engineering, biomimetics now is emerging in tribology.
•
Velcro was inspired from the structure of the hooking devices found on the fruit part of the plant
Galium aparine.
•
The outer surface of the Cathay Pacific Airbus 340, modeled after the grooved scales of shark skin, reduces drag and improves fuel efficiency.
The study and simulation of biological systems with desired properties is popularly known as biomimetics. Such an approach involves the transformation of the underlying principles discovered in nature into manmade technology.
Biomimetics, already popular in the fields of materials science and engineering, is being applied in diverse areas ranging from micro/nano-electronics to structural engineering and now is emerging in tribology. In this article, we’ll present examples of bio-inspired research works closely related to the field of tribology. In addition, examples of bio-inspired inventions also are given, with the view that it would create interest and appreciation in the readers.
VELCRO
Invented by George de Mestral and patented in 1955, Velcro is perhaps the earliest, most successful and popular of all the man-made inventions based on nature’s architecture. The design of the Velcro fastener was inspired from the structure of the hooking devices found on the fruit part of the plant,
Galium aparine (common names: Goosegrass, Spring Cleavers, Stickywilly) (
1).
When these fruits come in contact with foraging wildlife, they hook themselves onto the animal’s hairs and get interlocked. The fruit is then transported by the animals some distance away from the plant.
A Velcro fastener has two co-opted surfaces (
1), in which one surface is covered by stiff nylon hooks and the other by soft adaptive loops. When these two parts are pressed together, they get securely attached. In this hook-like frictional system, separation of the two parts requires a force in the form of strong jerks. The present-day Velcro fastener forms the basis of a multimillion dollar industry. As it can withstand hundreds of cycles of attachment/detachment operations, Velcro has good durability and is therefore a very useful and reusable invention.
TIRE TREAD EFFECT
Another of man’s creations analogous to a principle found in nature, namely the distinctive patterns on a tree frog’s foot, is the design of treads on automobile tires. Tree frogs such as
Amolops sp. possess large disc-like pads at the tip of their toes that assist them in attaching to surfaces such as leaves. The pads consist of flat-topped cuboidal columnar cells, which are separated from each other by canal-like spaces (
1). During climbing, water gets squeezed out from the contact through the channels between the foot and the surfaces on which they climb, making a perfect van der Waals contact.
Automobile tires have treads on them. While driving on wet roads, water flows out through the channels found between the treads, giving rise to intimate contact between the treads and the road, thereby creating sufficient grip during motion. This effect is known as the “Tire Tread Effect.”
GECKO EFFECT
Creatures such as beetles, flies, spiders and lizards have the ability to attach themselves to surfaces without falling off, even when the surfaces are vertically inclined. The presence of micro/nanostructures—small hairs called “setae” on their attachment pads—enables these creatures to attach and detach easily over any surface. As the mass of the creature increases, the radii of the terminal attachment structures decrease while the density of the structures increase (
2). The gecko is the largest animal that has this kind of dry attachment system and, therefore, is the main interest for scientific research.
In functional terms, the tiny hairs found on gecko feet (
3) are able to conform to the shape of surface irregularities to which the gecko is adhering. By mimicking the shape and geometry of gecko setae, synthetic adhesives have been made from polymeric materials. An example is “Gecko Tape (
4),” which can be used for several detachment-attachment cycles before the degradation of its adhesive property. This tape has arrays of flexible polyimide pillars fabricated using electron-beam lithography and dry etching in oxygen.
To create a gecko adhesive, the pillars must be sufficiently flexible and placed on a soft, flexible substrate so that individual tips can act in unison and attach to uneven surfaces all at the same time. To demonstrate the effectiveness of the gecko tape as a dry adhesive, a Spider-man toy was attached to a glass plate through the microfabricated gecko tape (
4). Polyurethane elastomer microfiber array with flat spatulate tips was fabricated by molding a master template using deep reactive ion etching, and the notching effect also can act as effective adhesive surfaces (
5). These surfaces, when tested against a smooth glass hemisphere for their adhesive property, show three times higher adhesion than flat polymeric surfaces.
Recently, scientists produced a super-adhesive surface called “Geckel (
6)” that is based on the surfaces of geckos and mussels. Using electron-beam lithography, they first created arrays of polymeric nanopillars and subsequently coated them with a polymer modeled on an amino acid, which is one of the building blocks of the “glue” protein in mussels. The result (gecko plus mussel) was named Geckel. This adhesive material has been tested to stick through 1,000 contact/release cycles and remains highly adhesive even underwater. By studying the structure of gecko setae, researchers also have designed high friction surfaces (
7) showing an increase in friction coefficient by more than four times.
Figure 1. (a) Hooking devices found on the fruit part of the plant, Galium aparine, (b) a magnified image of the hooking device (1), (c) the two co-opted surfaces of a Velcro fastener (1), (d) a tree frog, (e) schematic of flat-topped cuboidal columnar cells separated by canal-like spaces in tree frogs (Amolops sp.)1, (f) a close-up view of treads on a car tire.
SHARK SKIN EFFECT
Friction between a solid surface and a fluid also can be considered a tribological phenomenon. Inspiration from aquatic animals’ surface material and texture would benefit the design of surfaces that could increase efficiency in cases such as underwater navigation.
One such example is the “Shark Skin Effect.” Sharks’ skin has grooved scales on its entire body. The scales are directed almost parallel to the longitudinal body axis of the shark. The presence of this non-smooth surface texture on the shark skin effectively reduces drag by 5%-10%. Swimsuits with biomimetically designed surfaces that mimic the non-smooth surface texture on the shark skin have proved to be faster than conventional suits, as they reduce drag along key areas of the body.
Surface texture such as those on sharks’ skin also helps to reduce the friction between a solid surface and air. A transparent plastic film with similar microscopic texture (ribs parallel to the direction of flow) reduces aircraft drag by about 8% and is effective in saving fuel by about 1.5% (
8). The commercial aircraft Cathay Pacific Airbus 340 already has been fitted with ribbed structures on its body surface (
8).
Another interesting example is snake scales. Studying the frictional surfaces of snake scales would benefit when designing surfaces with anisotropic frictional characteristics. Snakes have friction-modifying structures consisting of ordered double-ridge microfibrillar array (
1). The double-ridge microfibrillar geometry provides significant reduction in adhesive forces, thereby creating ideal conditions for sliding in forward direction with minimum adhesion. Meanwhile, the highly asymmetric profile of the microfibrillar ending induces frictional anisotropy, as it acts as a locking mechanism prohibiting backward motion. Snake skin also has micropores that deliver an anti-adhesive lipid mixture, which further facilitates easy motion owing to boundary lubrication (
1).
Figure 2. (a)-(d) Terminal elements found on the attachment pads of various insects and gecko (2). As the size (mass) of the creature increases, the radius of the terminal attachment structures decreases, while the density of the structures increases, (e) Gecko, (f) SEM image of gecko setae (3).
LOTUS EFFECT
A number of plants have water-repellent leaves, which exhibit superhydrophobic property. Lotus (
Nelumbo nucifera) is the most popular example (water contact angle ~ 162 degrees (
9)). The unique ability of Lotus leaf surface to avoid wetting, popularly known as the “Lotus Effect,” is mainly due to the presence of microscale protuberances covered with waxy nanocrystals on their surface. Colocasia (
Colocasia esculenta) is another example of a plant whose leaves are superhydrophobic in nature (water contact angle ~ 164 degrees (
9)), due to the presence of micron-sized protuberances and wax crystals.
Both the protuberances and the wax crystals make the surfaces of Lotus and Colocasia leaves superhydrophobic in nature, which means water droplets easily roll over leaf surfaces taking contaminants and dust particles with them. This phenomenon is popularly known as the “Self-Cleaning Effect.” The self-cleaning property is highly important for water plants. In their habitats, these plants observe the presence of free water, which supports pathogenic organisms. These plants protect themselves from water-borne infections by hindering any adhesion of water necessary for the germination of pathogens (
9). Another reason for water-repellency is the fact that CO
2 diffuses 10% times slower in water than in air. The presence of water-repellent surface ensures that sufficient intake of CO
2 is observed for photosynthesis (
9).
Figure 3. (a) Shark, (b) riblets and grooves found on shark skin (8), (c) a swimming-cloth with similar surface as a shark skin could reduce frictional resistance, (d) an aircraft coated with a plastic film that has similar microscopic texture as found on a shark skin (8).
By studying the surface morphologies of water-repellent leaves, tribologists design and create hydrophobic surfaces to reduce adhesion between surfaces at small scales, which arise due to water condensation. Scientists and engineers are aware of the fact that surface forces such as adhesion and friction significantly oppose easy motion between tiny elements in miniaturized devices such as micro/nanoelectromechanical systems (MEMS/NEMS).
Minimizing the surface forces such as adhesion and friction, and also the occurrence of wear in miniaturized devices, is a real challenge, as the size of these devices is extremely small (usually their sizes are smaller than insects such as dust mites). Among the various attractive forces that contribute to adhesion at small-scales, the capillary force that arises due to the condensation of water from the environment is the strongest. Hence, there arises a need to modify surfaces at nano/microscale in order to achieve increased hydrophobicity that would drastically reduce adhesion due to capillary force, which in turn would also reduce friction.
Figure 4. (a) Lotus (Nelumbo nucifera), (b) protuberances on the surface of a Lotus leaf (11), (c) a micro-electro-mechanical system that has six gear chains, (d) the same MEMS device in comparison with the size of a dust mite, (e) nano-patterns that mimic the protuberances of a Lotus leaf (10), (f) Lotus-like (fresh) surface (11), (g) Colocasia-like (fresh) surface (12), (h) Colocasia-like (dry) surface (12).
Miniaturized devices such as MEMS/NEMS traditionally are made from silicon. The higher interfacial energy (hydrophilic nature) of silicon makes it a poor tribological material. Therefore, silicon surfaces need to be treated chemically/topographically to enhance their tribological performance.
Inspired by the intriguing surface structures/protuberances of the Lotus leaf that give rise to a superhydrophobic surface, nanoscale patterns that mimic the protuberances recently have been fabricated on thin polymeric films coated on silicon wafers, using a capillarity-directed soft lithographic technique (
10). These nanopatterned surfaces are hydrophobic in nature (water contact angle ~ 99 degrees) when compared to a bare silicon wafer (water contact angle ~ 22 degrees).
Investigation on adhesion and friction properties at the nanoscale has revealed that these surfaces exhibit superior tribological properties when compared to bare silicon flat surfaces. With the increased hydrophobicity and the reduced real area of contact projected by them, the nano-patterned surfaces exhibit adhesion and friction values that are lower by an order of magnitude than those of the bare silicon flat surfaces. Based on the published results, these nano-patterned polymeric surfaces with remarkable nanotribological properties have been recognized as prospective surfaces for tribological application in MEMS/NEMS.
Figure 5. (a) A sandfish that lives in the Sahara desert, (b) scales on the sandfish’s body (13), (c) mollusk shells (conchs) that are usually found on sandy beaches, (d) bivalve shells, (e) a pangolin with a layer of scales on its body, (f) a photograph of a pangolin scale (14).
Effective tribological surfaces also have been created by the direct replication of natural leaves of water-repellent plants (
11,
12). Indeed, this could be the first biomimetic approach of creating effective tribological surfaces by the direct replication of natural surfaces. Surfaces of Lotus and Colocasia leaves were replicated using the real surfaces of the leaves as natural templates. The Colocasia leaf surface was replicated both in its fresh and dried conditions, as it exhibits a significant change in its morphology upon drying, unlike the Lotus whose protuberances only shrink in size when drying.
In the case of the Colocasia leaf, the bumpy protuberances in its fresh condition become depressions upon drying, leaving behind a bump at the center and a ridge that surrounds each bump. It was found that the replicated surfaces: Lotus-like surface (
11), Colocasia-like (fresh) surface (
12) and Colocasia-like (dry) surface (
12) are hydrophobic in nature (water contact angles > 90 degrees). Results from microfriction tests reveal that the replicated surfaces exhibit friction coefficients almost four times lower than that of the bare silicon flat surfaces and, therefore, could be applied in MEMS application.
Figure 6. Schematic of typical joint with compressive load deforming the cartilage.
WEAR-RESISTANT SURFACES
Man has begun to learn from nature to design materials and textures that have superior wear-resistance characteristics. For example, a sandfish, living in the Sahara desert moves rapidly over the desert sand, and the scales on its body (
13) have excellent sand erosion wear resistance. Erosion experiments conducted using sand on the sandfish’s scales, glass and soft steel for 10 hours showed that the wear trace on the sandfish’s skin was the smallest, which suggests that its wear resistance is comparatively much higher than glass and soft steel (
13). The biomaterial comprising the sandfish’s scales and their surface texture together contribute toward its high wear resistance.
Mollusk (conch) shells experience water-sand erosion on sandy beaches. Their antiwear mechanism arises from the combination of their biotissues and their unique shape, which together prevent abrasion. The biomaterial of mollusk shells is a bioceramic composite that has a complex microstructure, the result of a billion years of evolution.
Understanding the formation and the microstructure of these bioceramic composites will help in the design of ceramics with superior mechanical properties such as toughness. Current methods used to fabricate ceramics still are unable to control parameters such as crystal density, orientation and morphological uniformity to the degree of perfection nature has achieved in mollusk shells. Studies on the microstructure of bivalve shells are expected to provide guidelines in developing biomimetic composite materials with better tribological properties.
Another interesting example is pangolin scales. A pangolin is a soil-burrowing animal with a layer of scales covering its body. As the pangolin burrows into soil, its scales are subjected to wear. A study on the chemical constitution of pangolin scales (
14) revealed the presence of 18 amino acids. The protein in the scales mainly consists of α-keratin and β-keratin. The specific elongation of pangolin scales is about 15% due to the presence of the proteins. Therefore, the plasticity of the pangolin scales is low, resulting in anti-abrasive characteristics (
14). Studies on biomaterials that exhibit remarkable friction and wear characteristics would provide insights toward enhancing the performance of soil-engaging engineering components such as those in agricultural machinery and earth moving machinery. A more detailed overview on the experimental biomimetic research works can be found in ref. [
15].
Figure 7. Schematic of possible self-adapting surface structures to cause controlled deformation.
THEORETICAL MODELING
For biological surfaces to be fully utilized in new technologies, the fundamental physical mechanisms causing their unique behaviors must be understood. By creating theoretical models and then correlating the models to the experimental observations of biomimetic surfaces, these mechanisms can be mathematically characterized and confirmed. In addition, the models can be used to optimize the surfaces for a specific application or goal.
Researchers have used existing tribological models and theories to consider biomimetic surfaces, even though they are very different from conventional tribological surfaces. Since the fundamental mechanics governing the surfaces are the same, these theories usually perform very well. For instance, researchers have developed models of adhesion of a gecko’s feet against a rough surface using classical elastic beam theory and rough surface contact. This model was then used to design an optimal surface array based on the gecko feet adhesion mechanism. In 2006 Tian and others found that during attachment and detachment the gecko can control the adhesion by several orders of magnitude by changing the motion of the foot. This mechanism was also optimized for practical use in new adhesive tapes.
In contrast to previous works which sought to model the highly adhesive nature of gecko feet, others have modeled the highly hydrophobic nature of some plant leaf surfaces (such as the previously discussed lotus leaf). Nosonovsky and Bhushan at Ohio State University modeled the wetting properties of surfaces with different surface profiles and structures that mimicked those of plants to obtain theoretically hydrophobic surfaces. These models are based on fundamental models used to describe the meniscus and surface tension of water particles such as the well-known Kelvin equation. The model can then be used to optimize surface design.
The design of lubricated bearings also can benefit greatly from our knowledge of the mechanisms at work in biological joints. Biological joints are very different than conventional industrial bearings. Friction, wear and lubrication of surfaces greatly affect the reliability and efficiency of the various joints in the human anatomy. When these joints fail, such as with severe osteoarthritis, the joints may require surgical repair or replacement.
However, the materials (metals, ceramics and polymers) used in most artificial joints are much different than the actual biomaterials in a human joint and are more closely related to industrial bearings. A typical joint (
16) usually has a softer layer of cartilage and other materials separating the hard bone. As discussed by Drs. Eddy Tysoe and Nic Spencer in the April 2006 issue of TLT, natural joints use soft material much more liberally than hard material, and perhaps it is to their advantage. More recent approaches use softer materials that are more similar to the original tissue such as hydrogels and actual living cells to replace joint surfaces.
A large number of works have numerically modeled the elastohydrodynamic lubrication of natural joints. Even then, the fundamental mechanical models used are essentially the same ones used to consider industrial hydrodynamic bearings and EHL in rolling element bearings. Most works successfully use the traditional theory of elasticity and Reynolds equation to consider hydrodynamic lubrication. Some models also incorporate the use of contact mechanics to calculate the friction, wear and stresses in the joints. Biomimetic modeling of bone also has been used to obtain materials with unique properties for hip implants.
Recently, numerical modeling has been used to design prototype surfaces designed to be soft and deformable like biological joints. These biomimetic self-adapting surfaces change their surface profiles at the nano- and microscale to improve performance. Using a built-in, closed-loop control system, the surfaces deform to provide additional load support as needed. The control system is created by the coupled elastic and hydrodynamic mechanisms without computerized or electronics control. The mechanism is similar to that seen in gas foil bearings.
Theoretical modeling has been used when studying the locomotion of animals such as water insects and snakes. Some insects and animals use surface tension to travel over water (water-walking) rather than through it. By using fundamental fluid mechanics, researchers were able to characterize this motion into different regimes based on the mechanism used to generate propulsion (
17). They found that small creatures are usually supported by surface tension while larger creatures tend to use momentum and “water slapping.” Perhaps these same mechanisms could be harnessed to create bearings that use surface tension to carry load.
Figure 8. Characterization of animal “water walking” in terms of non-dimensional quantities.
Based on this survey, it appears that traditional mechanical models can be used to characterize and explain biological mechanisms. The difficulty is obtaining accurate material properties and models that describe the often unusual biological materials encountered. Therefore these same models also can be used to optimize biomimetic surfaces before prototypes are fabricated.
REFERENCES
This article references work from more than 70 technical papers. For the complete list, contact any of the three authors.
1.
Scherge, M. and Gorb, S.N. (2001),
Biological Micro- and Nanotribology, Springer-Verlag, Berlin Heidelberg.
2.
Arzt, E., Gorb, S., and Spolenak, R. (2003), From Micro to Nano Contacts in Biological Attachment Devices,
Proceedings of National Academy of Sciences (PNAS),
100 (19), p. 10603.
3.
Naik, R.R. and Stone, M.O., “Integrating Biomimetics,”
Materials Today,
18, Sept. 2005.
4.
Geim, A.K., Dubonos, S.V., Grigorieva, I.V., Novoselov, K.S., Zhukov, A.A., and Shapoval, S.-Y. (2003), “Microfabricated Adhesive Mimicking Gecko Foot-Hair,”
Nature Materials,
2, p. 461.
5.
Kim, S. and Sitti, M. (2006), “Biologically Inspired Polymer Microfibers with Spatulate Tips as Repeatable Fibrillar Adhesives,”
Applied Physics Letters,
89, p. 261911-1.
6.
Lee, H., Lee, B.P., and Messersmith, P.B. (2007), “A Reversible Wet/Dry Adhesive Inspired by Mussels and Geckos,”
Nature Letters,
448, p. 338.
7.
Bhushan, B. and Sayer, R.A. (2007), “Surface Characterization and Friction of a Bio-Inspired Reversible Adhesive Tape,”
Microsystems Technology,
13, p. 71.
8.
Ball, P. (1999), “Shark Skin and Other Solutions,”
Nature,
400, p. 507.
9.
Neinhuis, C. and Barthlott, W. (1997), “Characterization and Distribution of Water-Repellent, Self-Cleaning Plant Surfaces,”
Annals of Botany,
79, p. 667.
10.
Yoon, E.-S., Singh, R.A., Kong, H., Kim, B., Kim, D.-H., Jeong, H.E., and Suh, K.Y. “Tribological Properties of Bio-Mimetic Nano-Patterned Polymeric Surfaces on Silicon Wafer,”
Tribology Letters,
21 (1), p. 31-37.
11.
Singh, R.A., Yoon, E.-S., Kim, H.J., Kong, H., Park, S., Jeong, H.E., and Suh, K.Y. (2007), “Enhanced Tribological Properties of Lotus Leaf-Like Surfaces Fabricated by Capillary Force Lithography,”
Surface Engineering,
23 (3), p. 161.
12.
Singh, R.A., Yoon, E.-S., Kim, H.J., Kim, J., Jeong, H.E., and Suh, K.Y. (2007), “Replication of Surfaces of Natural Leaves for Enhanced Micro-Scale Tribological Property,”
Materials Science & Engineering C: Biomimetic and Supramolecular Systems,
27, p. 875.
13.
Rechenberg, I. (2003), “Tribological Characteristics of Sandfish,” in Nature as Engineer and Teacher: Learning for Technology from Biological Systems, Shanghai, Oct. 8-11.
14.
Tong, J., Ma, Y.-H., Ren, L.-Q., and Li, J.-Q. (2000), “Tribological Characteristics of Pangolin Scales in Dry Sliding,”
Journal of Materials Science Letters,
19, p. 569.
15.
Singh, R.A. and Yoon, E.-S. (2008), “Biomimetics in Tribology—Recent Developments,”
Journal of the Korean Physical Society,
52 (3), p. 661.
16.
Mansour, J.M. (2003), “Chapter 5: Biomechanics of Cartilage,” in Kinesiology: The Mechanics and Pathomechanics of Human Movement, C.A. Oatis, Ed. Philadelphia: Lippincott Williams and Wilkins, p. 66.
17.
Bush, J.W.M. and Hu, D.L. (2006), “Walking on Water: Biolocomotion at the Interface,”
Annual Review of Fluid Mechanics,
38, p. 339.
Dr. R. Arvind Singh
Dr. Eui-Sung Yoon
Dr. Robert L. Jackson
Dr. R. Arvind Singh is a visiting scientist at the Nano-Bio Research Center, Korea Institute of Science and Technology (KIST), Seoul, South Korea arvindsingh.r@gmail.com.
Dr. Eui-Sung Yoon is the director of the Nano-Bio Research Center, Nano-Science Research Division, Korea Institute of Science and Technology (KIST), Seoul, South Korea esyoon@kist.re.kr.
Dr. Robert L. Jackson is an assistant professor in mechanical engineering at Auburn University in Alabama robert.jackson@eng.auburn.edu.