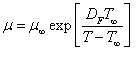
And the Johari and Whalley equation represents the pressure dependence.
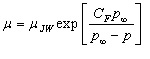
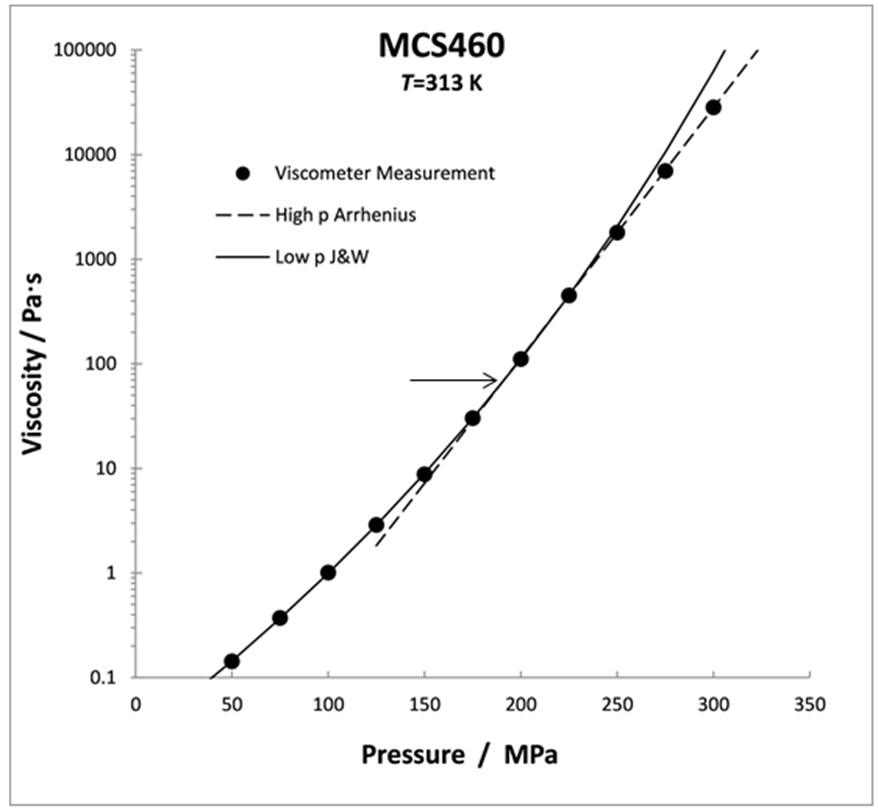
Figure 2. The pressure dependence at 313 K showing a crossover (at the horizontal arrow) which can be best represented by two J&W equations.
DISCUSSION: With the recent trend toward the use of real viscosity in analyses, the possibility of a glass transition in the lubricant film cannot be overlooked. This event is more likely for the liquids designed for high friction, traction fluids, which are also susceptible to a dynamic crossover. The glass transition is a phenomenon occurring at a specific value of viscosity for a given liquid. It seems well-established that for the much studied inorganic liquids (mineral melts) the glass transition viscosity is of the order of 1012 Pa·s. However, the situation for organic liquids is less clear. Here, for one liquid lubricant, the glass transition by dilatometry combined with falling cylinder viscometry places the viscosity at the transition at between 107 and 108 Pa∙s, in the same range as squalane.
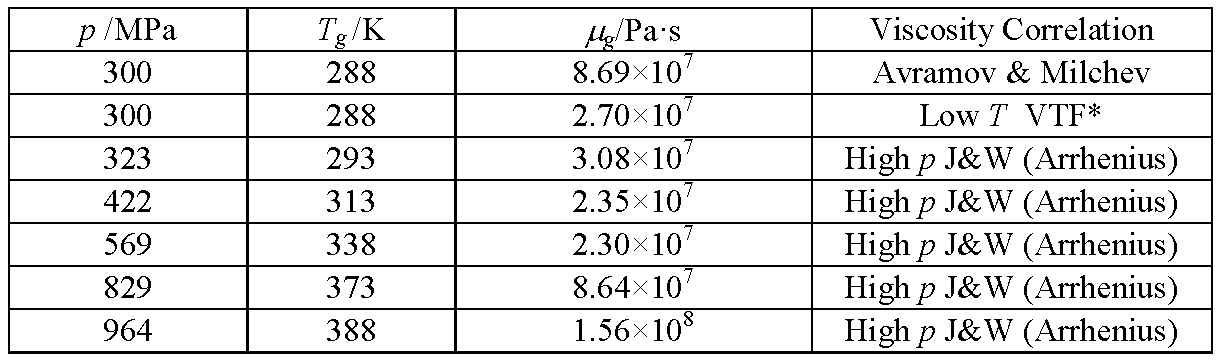
REFERENCES:
1. Yasutomi, S., Bair, S., & Winer, W. O. (1984). An application of a free volume model to lubricant rheology I—dependence of viscosity on temperature and pressure. Journal of tribology, 106(2), 291-302.
2. Schweyer, H. E. (1974). Glass transition of asphalts under pressure. Journal of Testing and Evaluation, 2(1), 50-56.
3. Deegan, R. D., Leheny, R. L., Menon, N., Nagel, S. R., & Venerus, D. C. (1999). Dynamic shear modulus of tricresyl phosphate and squalane. The Journal of Physical Chemistry B, 103(20), 4066-4070.
4. Bair, S. S., Andersson, O., Qureshi, F. S., & Schirru, M. M. (2017). New EHL Modeling Data for the Reference Liquids Squalane and Squalane plus Polyisoprene. Tribology Transactions, DOI: 10.1080/10402004.2017.1310339.
5. Oels, H. J., & Rehage, G. (1977). Pressure-Volume-Temperature Measurements on Atactic Polystyrene. A Thermodynamic View. Macromolecules, 10(5), 1036-1043.